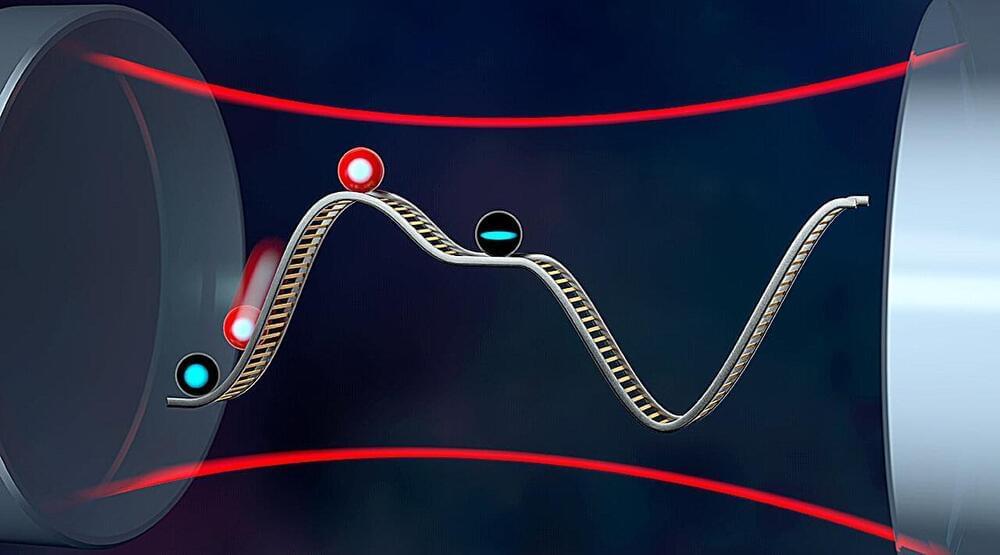
While atomic clocks are already the most precise timekeeping devices in the universe, physicists are working hard to improve their accuracy even further. One way is by leveraging spin-squeezed states in clock atoms.
Spin-squeezed states are entangled states in which particles in the system conspire to cancel their intrinsic quantum noise. These states, therefore, offer great opportunities for quantum-enhanced metrology since they allow for more precise measurements. Yet, spin-squeezed states in the desired optical transitions with little outside noise have been hard to prepare and maintain.
One particular way to generate a spin-squeezed state, or squeezing, is by placing the clock atoms into an optical cavity, a set of mirrors where light can bounce back and forth many times. In the cavity, atoms can synchronize their photon emissions and emit a burst of light far brighter than from any one atom alone, a phenomenon referred to as superradiance. Depending on how superradiance is used, it can lead to entanglement, or alternatively, it can instead disrupt the desired quantum state.